Dynamics of cellobiose dehydrogenase
SUPERVISOR: CHRIS OOSTENBRINK
Background.
The structure and function of complex macromolecular entities, like proteins, polymers or mineral structures have been studied by molecular simulation and led to insight into the molecular mechanisms that underlie experimental observations (Karplus and Kuriyan, 2005; van Gunsteren et al., 2006). Two aspects are commonly distinguished that play crucial roles to get meaningful results: sampling and scoring. Sampling involves the generation of a sufficiently large amount of molecular structures to describe the orientations and conformations that in reality lead to the observed (average) quantities that are measured. These may be obtained from a variety of search techniques, like systematic searches, molecular dynamics or monte carlo simulations. Scoring, on the other hand, is required to evaluate how likely the generated conformations are in terms of energy and to discriminate ‘good’ onformations from ‘bad’ conformations. The assignment of an energy value to a molecular conformation can again be done in a variety of ways, ranging from advanced quantum mechanical calculations, through force fields to empirical, or knowledge-based scoring functions.
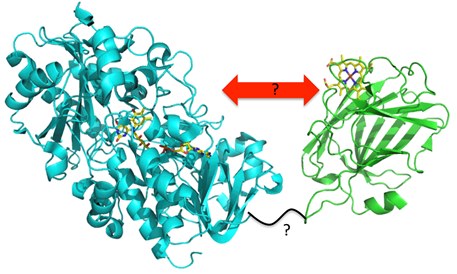
In this project we aim to use computational methods to study enzymes that play a role in the newly proposed oxidative breakdown of cellulose, in close collaboration with experiments performed by HALTRICH. Cellobiose dehydrogenase (CDH) is an extracellular, fungal enzyme of ca. 90 kDa, which consists of an N-terminal cytochrome domain connected by a flexible linker to a C-terminal flavodehydrogenase domain (Henriksson et al., 2000). It was observed that CDH can generate reduction equivalents and transport it to other redox enzymes such as lytic polysaccharide monooxygenase (LPMO) or Cytochrome C, but direct electron transfer to electrodes has also been described (Tasca et al., 2009; Coman et al., 2008; Sygmund et al., 2012) The proposed natural function of the cytochrome domain of CDH is to transfer electrons to LPMOs, which are involved in the breakdown of crystalline cellulose regions (Phillips et al., 2011; Langston et al., 2011; Kittl et al., 2012). The intramolecular interaction between the flavin and heme domains of CDH appears to be governed by a subtle equilibrium and is hence expected to strongly influence the efficiency of the electron transfer reactions. In order to fully understand the electron transfer processes, the atomic and possibly electronic degrees of freedom need to be taken into account, while an appropriate description of the interdomain interaction requires extensive sampling of the conformational space. In order to quantify the equilibrium between the closely interacting poses of the domains and poses in which the domains are further removed, the interdomain interactions needs to be accurately scored.
Aims and methods.
In this project, we will study the electron transfer between the two domains in CDH and further transfer to partner proteins or electrodes. We have recently successfully described the electron transfer paths in Cytochrome P450 reductase, another multidomain electron transfer protein. In this protein, the electron transfer strongly depends on the conformation of the reductase, displaying an open and a closed state (Suendermann and Oostenbrink, 2013). The X-ray structures of both open and closed forms of intact CDH have not been published yet, but have been solved and are already available to us. The flexible linker between the flavin and heme domains will pose additional challenges on the sampling, for which enhanced sampling methods may be further developed. In particular, we plan to use the distance-field reaction coordinate, which we developed recently (De Ruiter and Oostenbrink, 2013), in conjunction with either Hamiltonian replica exchange simulations or with local elevation. The effect of protein dynamics on the efficiency of the electron transfer pathway will be studied. The electron transfer can be described classically using decay rates for different types of connections between atoms (Beratan et al., 1990) and the most likely paths can be obtained using Dijkstra′s algorithm (Dijkstra, 1959). By performing this analysis on a large set of protein conformations the dynamic fluctuations in the electron transfer may be determined.
By constructing specific mutants that may influence the proposed electron transfer path or the binding equilibrium between the two domains, the suggested function of the amino acids may be confirmed both experimentally and computationally. While the experiments will be performed by HALTRICH and OBINGER, we will create models of the mutants and explore the possibilities of mutated residues to be involved in protein stability, electron transfer, the interactions between the domains or interactions between CDH and its electron transfer partners. The interactions between CDH and small-molecule substrates, electron transfer partners or electrodes will be studied by automated (protein-protein) docking experiments. Successful docking studies of cellobiose and a series of analogues have already been described previously (Mulla et al., 2013). To predict complexes of CDH with LPMO, we will use state of the art protein-protein docking experiments (Dominguez et al., 2003, De Vries et al., 2007).
Beratan, D.N., Onuchic, J.N., Betts, J.N., Bowler, B.E., Gray, H.B. (1990) Electron tunneling pathways in ruthenated proteins. J. Am. Chem. Soc. 112, 7915–7921
de Ruiter, A., Oostenbrink, C. (2013) Protein-ligand binding from distancefield distances and Hamiltonian replica exchange simulations. J. Chem. Theory Comp. 9, 883-892
de Vries, S.J., van Dijk, A.D.J., Krzeminski, M., van Dijk, M., Thureau, A., Hsu, V., Wassenaar, T., Bonvin, A.M.J.J. (2007) HADDOCK versus HADDOCK: New features and performance of HADDOCK2.0 on the CAPRI targets. Proteins 69, 726-733
Dominguez, C., Boelens, R., Bonvin, A.M.J.J. (2003) HADDOCK: A protein−protein docking approach based on biochemical or biophysical information. J. Am. Chem. Soc. 125, 1731-1737
Dijkstra, E.W. (1959) A note on two problems in connexion with graphs. Numer. Mathem. 1, 269–271
Coman, V., Vaz-Dominguez, C., Ludwig, R., Harreither, W., Haltrich, D., De Lacey, A. L., Ruzgas, T., Gorton, L., Shleev, S. (2008) A membrane-, mediator-, cofactor-less glucose / oxygen biofuel cell. Phys. Chem. Chem. Phys. 10, 6093-6096
Henriksson, G., Johansson, G., Pettersson, G. (2000) A critical review of cellobiose dehydrogenases. J. Biotechnol. 78, 93-113
Karplus, M., Kuriyan, J. (2005) Molecular dynamics and protein functions. Proc. Nat. Acad. Sci. USA 102, 6679-6685
Kittl, R., Kracher, D., Burgstaller, D., Haltrich, D., Ludwig, R. (2012) Production of four Neurospora crasse lytic polysaccharide monooxygenases in Pichia pastoris monitored by a fluorimetric assay. Biotechnol. Biofuels 5:79
Langston, J.A., Shaghasi, T., Abbate, E., Xu, F., Vlasenko, E., Sweeney, M.D. (2011) Oxidoreductive cellulose depolymerization by the enzymes cellobiose dehydrogenase and glycoside hydrolase 61. Appl. Environ. Microbiol. 77, 7007–7015
Mulla, D., Kracher, D., Ludwig, R., Nagy, G., Grandits, M., Holzer, W., Saber, Y., Gabra, N., Viernstein, H., Unger, F.M. (2013) Azido derivatives of cellobiose: oxidation at C1 with cellobiose dehydrogenase from Sclerotium rolfsii. Carbohydr. Res. 382, 86–94
Phillips, C.M., Beeson, W.T., Cate, J.H., Marletta, M.A. (2011) Cellobiose dehydrogenase and a copper-dependent polysaccharide monooxygenase potentiate cellulose degradation by Neurospora crassa. ACS Chem. Biol. 6, 1399–1406
Suendermann A., Oostenbrink, C. (2013) Molecular dynamics simulations give insight into the conformational change, complex formation, and electron transfer pathway for cytochrome P450 reductase. Protein Sci. 22, 1183–1195
Sygmund, C., Kracher, D., Scheiblbrandner, S., Zahma, K., Felice, A.K.G., Harreither, W., Kittl, R., Ludwig, R. (2012) Characterization of the two Neurospora crassa cellobiose dehydrogenases and their connection to oxidative cellulose degradation. Appl. Environ. Microbiol. 78, 6161-6171
Tasca, F., Gorton, L., Harreither, W., Haltrich, D., Ludwig, R., Noell, G. (2009) Comparison of direct and mediated electron transfer for cellobiose dehydrogenase from Phanerochaete sordida. Anal. Chem. 81, 2791-2798
van Gunsteren, W. F., Bakowies, D., Baron, R., Chandrasekhar, I., Christen, M., Daura, X., Gee, P., Geerke, D., Glättli, A., Huenenberger, P. H., Kastenholz, M. A., Oostenbrink, C., Schenk, M., Trzesniak, D., van der Vegt, N. F. A., Yu, H. B. (2006) Biomolecular modeling: goals, problems, perspectives. Angew. Chem. Int. Ed. 45, 4064-4092