Increasing thermostability of pyranose oxidase by enzyme evolution
PRINCIPAL INVESTIGATOR: Dietmar HALTRICH
Background.
The tetrameric fungal enzyme pyranose oxidase (POx), a member of the GMC family of FAD-dependent oxidoreductases, catalyses the oxidation of several aldopyranoses at position C-2 while using electron acceptors such as O2, various quinones and metal ions (Sützl et al., 2018).
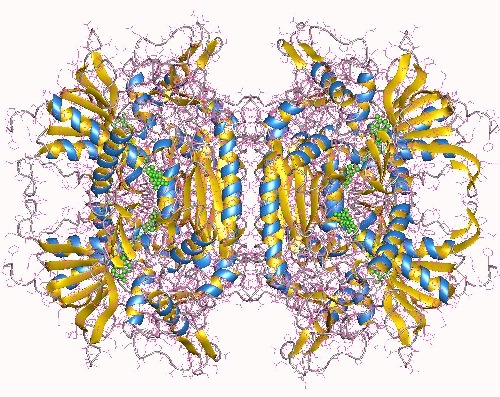
The excellent reactivity of POx with alternative electron acceptors can be exploited in applications such as biofuel cells or biosensors, as we have shown previously (Spadiut et al., 2010). Enzymatic biofuel cells (BFCs) convert e.g. sugars into electrical energy by employing oxidoreductases as the anodic bio-component and coupling this with a suitable enzyme on the cathode for O2 reduction. The transport of the electrons from the active site of the selected enzyme to the electrode can happen directly, which is however rarely encountered. In mediated electron transfer, certain mediators such as quinones, ferrocenes or Os redox polymers collect the electrons from the enzyme and transfer them to an electrode. BFCs have highly attractive applications, e.g., for the electrical activation of implantable electronic medical devices, etc. (Barton et al. 2004; Grattieri & Minteer, 2018).
Two main obstacles hampering the development of BFCs are the rather low current output as well as the insufficient stability of the biocomponents. Recently, we could show that engineered variants of POx from Trametes multicolor (TmPOx), which is currently the best studied member of the POx family, showed an energy output that was increased by a factor of up to eight compared to the wild-type enzyme (Spadiut et al. 2010). The increase in catalytic rates, however, came at the cost of stability of the variants. Initial studies in our group have shown that enzyme engineering can be employed to increase thermostability of TmPOx significantly (Spadiut et al., 2009).
Aims and methods.
It is the main aim of this project to employ different methods of directed evolution to improve the (thermo)stability of POx. Increased stability is not only of applied interest, but it has been shown that extra stability increases evolvability by allowing a protein to accept a wider range of beneficial mutations while still folding to its native structure, thus giving it mutational robustness as the basis for further engineering studies (Bloom et al., 2006).
We will use the approach of iterative saturation mutagenesis on the basis of B factors to increase stability. Systematic structural studies comparing mesophilic and thermophilic enzymes have shown that the latter are characterized by higher degrees of rigidity resulting from the accumulation of a variety of different and often subtle effects. Enhancing the thermostability of mesophilic enzymes should therefore be possible by increasing the rigidity at appropriate sites (Eijsink et al., 2005; Yu & Huang, 2014). The sites, at which rigidity is to be increased, will be selected on the basis of the B factor, with high B factors indicating pronounced degrees of thermal motion and thus flexibility. Sites chosen for TmPOx include e.g. residues 81–84 (glycine turn at the 2-fold interface between subunits A and B), the surface loops 184–188, 266–273, 336–347, or parts of the head domain 382–390 and 395–407. Additional regions of the protein that contribute to the first steps in unfolding will be identified by molecular modelling and simulations together with OOSTENBRINK. One or more amino acids of these sites will be selected for saturation mutagenesis with the formation of small focused libraries. The gene of the best hit is subsequently used as a template for a second round of saturation mutagenesis at another site and the process is continued by focusing on the remaining sites iteratively. This concept of “iterative saturation mutagenesis” is crucial for success, as the efficiency of the method is derived from the rapid build-up of evolutionary pressure (Reetz et al., 2006).
An alternative strategy for engineering increased stability will be the strengthening of the interactions between the subunits of POx; to this end, residues Q461, S113, and L537 to M541 will be targeted, amongst others, again in an iterative way using saturation mutagenesis. Additionally, a network of ion bonds will be rationally engineered at the subunit interface. Concomitantly, we will use an approach to weaken these subunit interactions, thereby obtaining stable and catalytically active dimers. These will have an active site that is more accessible and should give important information on the function of the rather restricted active site of POx. Proposed research work will include (i) detailed analysis of the structure of TmPOx and selection of target residues (together with OOSTENBRINK); (ii) preparation of mutational libraries using saturation mutagenesis; (iii) screening for increased stability using established well plate-based assays; (iv) detailed measurement of thermodynamic and kinetic stability of variant POx (determination of melting temperature, half-life of activity t1/2) (v) steady-state kinetic analysis of variant POx; (vi) determination of the crystal structure in collaboration with structural biologists.
Collaborations within this thesis will include OOSTENBRINK (molecular modelling and simulations).
Grattieri, M. and Minteer, S.D. (2018) Self-powered biosensors. ACS Sensors 3, 44-53. doi: 10.1021/acssensors.7b00818
Bloom, J.D., Labthavikul, S.T., Otey, C.R., Arnold, F.H. (2006) Protein stability promotes evolvability. Proc. Natl. Acad. Sci. 103, 5869–5874. doi:10.1073/pnas.0510098103
Barton, S.C., Gallaway, J., Atanassov, P. (2004) Enzymatic biofuel cells for implantable and microscale devices. Chem. Rev. 104, 4867-4886. doi: 10.1021/cr020719k
Eijsink, V.G., Gåseidnes, S., Borchert, T.V., van den Burg, B. (2005) Directed evolution of enzyme stability. Biomol. Eng. 22, 21–30. doi: 10.1016/j.bioeng.2004.12.003
Hallberg, B.M., Leitner, C., Haltrich, D., Divne, C. (2004) Crystal structure of the 270-kDa homotetrameric lignin-degrading enzyme pyranose 2-oxidase. J. Mol. Biol. 341, 781–796. doi: 10.1016/j.jmb.2004.06.033
Spadiut, O., Brugger, D., Coman, V., Haltrich, D., Gorton, L (2010) Engineered pyranose 2-oxidase: Efficiently turning sugars into electrical energy. Electroanalysis 22, 813-820. doi: 10.1002/elan.201800096
Spadiut, O., Leitner, C., Salaheddin, C., Varga, B., Vertessy, B.G., Tan, T.C., Divne, C., Haltrich, D. (2009): Improving thermostability and catalytic activity of pyranose 2-oxidase from Trametes multicolor by rational and semi-rational design. FEBS J. 276, 776–792. doi: 10.1111/j.1742-4658.2008.06823.x
Sützl, L., Laurent, C.V.F.P., Abrera, A.T., Schütz, G., Ludwig, R., Haltrich, D. (2018) Multiplicity of enzymatic functions in the CAZy AA3 family. Appl. Microbiol. Biotechnol. 102, 2477–2492. doi: 10.1007/s00253-018-8784-0
Yu, H., Huang, H. (2014) Engineering proteins for thermostability through rigidifying flexible sites. Biotechnol. Adv. 32, 308-315. doi: 10.1016/j.biotechadv.2013.10.012