Control of glycolysis in Crabtree-negative and positive yeasts
SUPERVISOR: DIETHARD MATTANOVICH
Background.
Baker’s yeast (Saccharomyces cerevisiae) stands out for an exceptionally high fermentative capacity which is triggered by high sugar supply, even in oxidative conditions. This phenotype, called the Crabtree effect, is supposed to have evolved in three main events (Dashko et al., 2014): (1) occurrence of overflow metabolism; (2) ability to grow anaerobically; (3) rewiring of the transcriptional network of sugar consumption. It is assumed that overflow metabolism was an outcome of a whole genome duplication (WGD) event during yeast evolution (Wolfe and Shields, 1997), however also some pre-WGD yeasts show a Crabtree-positive phenotype (Hagman and Piskur, 2015), indicating that overflow metabolism probably occurred prior to WGD. We recently found that in Komagataella phaffii (syn. Pichia pastoris), deregulation of a single gene encoding a transcription factor is sufficient to turn this canonical Crabtree-negative yeast into a fermenting, Crabtree-positive yeast (Ata et al., 2018). This transcription factor gene (CRA1) is orthologous to GAL4, however controlling glycolysis instead of galactolysis in K. phaffii (and probably other Crabtree-negative yeasts).
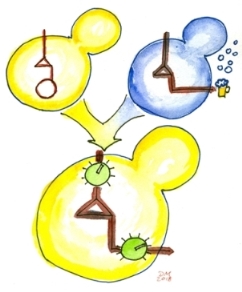
We hypothesize that transcriptional deregulation was an initial step in the evolution of Crabtree-positive yeasts, creating a first evolutionary advantage of overflow metabolism. This means that glycolysis is controlled at the transcriptional level, determining enzyme levels as the main flux-controlling determinant. In S. cerevisiae, however, glycolysis is mostly not regulated at the levels of transcription and enzyme level, but metabolically (Daran-Lapujade et al., 2007; van den Brink et al., 2008; Hackett et al., 2016). Therefore, it can be assumed that overflow metabolism came with a massive overproduction of glycolytic enzymes which pertains in Crabtree-positive yeasts. It is accepted today that the total cellular protein synthesis capacity is limited by the supply of precursor metabolites and energy (Carbonell-Ballestero et al., 2016; Noor et al., 2016; Borkowski et al., 2018), so that glycolytic overexpression would shift the proteome towards glycolytic enzymes, away from other metabolic enzymes. This re-allocation of resources will impact cell metabolism on many levels.
Aims and methods.
This project aims to answer following questions:
1. Which fraction of the proteome is occupied by glycolysis? The fraction of the total proteome of K. phaffii and S. cerevisiae allocated to glycolysis will be measured by targeted proteomics using synthetic peptides as standards (Pan et al., 2009). Three strains will be compared here: K. phaffii wild-type (Crabtree neg.), K. phaffii CRA1 overexpressing (Crabtree pos.), and S. cerevisiae wild-type (Crabtree pos). They will be cultivated in chemostat both at a low dilution rate (non-fermenting) and a high dilution rate (fermenting).
2. Are glycolytic enzymes of S. cerevisiae more efficient? As mentioned above, glycolysis is not regulated at the level of enzyme amounts (transcription and translation) in S. cerevisiae. The initial experiments will show whether the amount of glycolytic enzymes is always as high as in deregulated, Crabtree-positive K. phaffii. This would indicate that the maximum capacity of the S. cerevisiae enzymes is higher than in K. phaffii. We will investigate this hypothesis by transplanting glycolytic genes of S. cerevisiae into K. phaffii. One gene per reaction is sufficient to enable strong glycolytic flux in S. cerevisiae (Solis-Escalante et al., 2015). These ORFs will replace one by one the K. phaffii ORFs in their native loci, by employing Golden Gate cloning (Prielhofer et al., 2017) and CRISPR-Cas9 genome editing (Gassler et al., 2018). Enzyme activities and catalytic properties will be measured according to Jansen et al., 2005.
3. How is K. phaffii metabolism impacted by deregulation of glycolysis? The glycolytic flux in the CRA1 OE strain is more than 2-fold increased over the wild-type, while PPP and TCA fluxes are reduced (Ata et al., 2018). Using the proteome data and published flux data, the load of glycolytic enzyme synthesis will be modeled by Resource Balance Analysis (RBA) (Reimers et al., 2017; Goelzer et al., 2011) and kinetic modeling (Kesten et al., 2015).
Collaborations within this thesis will include ZANGHELLINI (metabolic modeling), ALTMANN (proteomics) and HANN (metabolomics).
Ata, Ö., Rebnegger, C., Tatto, N.E., Valli, M., Mairinger, T., Hann, S., Steiger, M., Çalık, P., Mattanovich, D. (2018) A single transcription factor activates the Crabtree effect in Komagataella phaffii. Nat. Commun., 9:4911. doi: 10.1038/s41467-018-07430-4
Borkowski, O., Bricio, C., Murgiano, M., Rothschild-Mancinelli, B., Stan, G.B., Ellis, T. (2018) Cell-free prediction of protein expression costs for growing cells. Nat. Commun. 9, 1457. doi: 10.1038/s41467-018-03970-x
Carbonell-Ballestero, M., Garcia-Ramallo, E., Montañez, R., Rodriguez-Caso, C., Macía, J. (2016) Dealing with the genetic load in bacterial synthetic biology circuits: convergences with the Ohm's law. Nucleic Acids Res. 44, 496-507. doi: 10.1093/nar/gkv1280
Daran-Lapujade, P., Rossell, S., van Gulik, W.M., Luttik, M.A., de Groot, M.J., Slijper, M., Heck, A.J., Daran, J.M., de Winde, J.H., Westerhoff, H.V., Pronk, J.T., Bakker, B.M. (2007) The fluxes through glycolytic enzymes in Saccharomyces cerevisiae are predominantly regulated at posttranscriptional levels. Proc. Natl. Acad. Sci. USA 104, 15753-15758. doi:10.1073/pnas.0707476104
Dashko, S., Zhou, N., Compagno, C., Piškur, J. (2014) Why, when, and how did yeast evolve alcoholic fermentation? FEMS Yeast Res. 14, 826-832. doi: 10.1111/1567-1364.12161
Gassler, T., Heistinger, L., Mattanovich, D., Gasser, B., Prielhofer R. (2018) CRISPR/Cas9-mediated homology directed genome editing in Pichia pastoris. Methods Mol. Biol., accepted
Goelzer, A. and Fromion, V. (2017) Resource allocation in living organisms. Biochem. Soc. Trans. 45, 945-952. doi: 10.1042/BST20160436
Hackett, S.R., Zanotelli, V.R., Xu, W., Goya, J., Park, J.O., Perlman, D.H., Gibney, P.A., Botstein, D., Storey, J.D., Rabinowitz, J.D. (2016) Systems-level analysis of mechanisms regulating yeast metabolic flux. Science 354, aaf2786. doi:10.1126/science.aaf2786
Hagman, A., Piškur, J. (2015) A study on the fundamental mechanism and the evolutionary driving forces behind aerobic fermentation in yeast. PLoS One 10, e0116942. doi: 10.1371/journal.pone.0116942.
Jansen, M.L., Diderich, J.A., Mashego, M., Hassane, A., de Winde, J.H., Daran-Lapujade, P., Pronk, J.T. (2005) Prolonged selection in aerobic, glucose-limited chemostat cultures of Saccharomyces cerevisiae causes a partial loss of glycolytic capacity. Microbiology 151, 1657-1669. doi:10.1099/mic.0.27577-0
Kesten, D., Kummer, U., Sahle, S., Hübner, K. (2015) A new model for the aerobic metabolism of yeast allows the detailed analysis of the metabolic regulation during glucose pulse. Biophys. Chem. 206, 40-57. doi: 10.1016/j.bpc.2015.06.010
Noor, E., Flamholz, A., Bar-Even, A., Davidi, D., Milo, R., Liebermeister, W., Beard, D.A. (2016) The Protein cost of metabolic fluxes: Prediction from enzymatic rate laws and cost minimization. PLoS Comput. Biol. 12, e1005167. doi: 10.1371/journal.pcbi.1005167
Pan, S., Aebersold, R., Chen, R., Rush, J., Goodlett, D.R., McIntosh, M.W., Zhang, J., Brentnall, T.A. (2009) Mass spectrometry based targeted protein quantification: methods and applications. J. Proteome Res. 8, 787-797. doi: 10.1021/pr800538n.
Prielhofer, R., Barrero, J.J., Steuer, S., Zahrl, R., Baumann, K., Gassler, T., Sauer, M., Mattanovich, D., Gasser, B., Marx, H. (2017) GoldenPiCS: A Golden Gate-derived modular cloning system for applied synthetic biology in the yeast Pichia pastoris. BMC Sys. Biol. 11, 123. doi: 10.1186/s12918-017-0492-3
Reimers, A.M., Lindhorst, H., Waldherr, S. (2017) A protocol for generating and exchanging (genome-scale) metabolic resource allocation models. Metabolites 7, E47. doi: 10.3390/metabo7030047.
Solis-Escalante, D., Kuijpers, N.G., Barrajon-Simancas, N., van den Broek, M., Pronk, J.T., Daran, J.M., Daran-Lapujade, P. (2015) Minimal set of glycolytic genes reveals strong redundancies in Saccharomyces cerevisiae central metabolism. Eukaryot. Cell 14, 804-816. doi: 10.1128/EC.00064-15
van den Brink, J., Canelas, A.B., van Gulik, W.M., Pronk, J.T., Heijnen, J.J., de Winde, J.H., Daran-Lapujade, P. (2008) Dynamics of glycolytic regulation during adaptation of Saccharomyces cerevisiae to fermentative metabolism. Appl. Environ. Microbiol. 74, 5710-5723. doi: 10.1128/AEM.01121-08
Wolfe, K.H. and Shields, D.C. (1997) Molecular evidence for an ancient duplication of the entire yeast genome. Nature 387, 708-713. doi:10.1038/42711