Engineering of pyranose oxidoreductases for modified oxygen reactivity
SUPERVISOR: DIETMAR HALTRICH
Project assigned to: IRIS KRONDORFER and DAGMAR BRUGGER
Background.
Flavin-dependent enzymes catalyze dehydrogenations and electron-transfer reactions, halogenations, light sensing and emissions, DNA repair and even protein folding and chromatin remodeling (Joosten and van Berkel, 2007, Mattevi, 2006). Flavoenzyme oxidoreductase reactions consist of two half-reactions. In the reductive half-reaction, the substrate reduces the flavin, and in the oxidative half-reaction, the (reduced) flavin is re-oxidized by an electron acceptor. Flavin-dependent oxidases use dioxygen as electron acceptor, producing hydrogen peroxide, whereas dehydrogenases and electron transferases react either very slowly or not at all with oxygen, and utilize alternative electron acceptors such as various (substituted) quinones or redox-proteins. The chemical and structural basis of oxygen reactivity remains largely undetermined (Mattevi, 2006), and a comparison of the active sites of flavin-dependent enzymes from various structural families and catalytic properties has not yielded any indications of a specific binding sites for either oxygen or alternative electron acceptors. Oxygen has been shown to be able to diffuse through a protein rather easily, or utilize internal cavities and tunnels (Leferink et al., 2009).
The FAD-dependent pyranose 2-oxidase (POx, glucose 2-oxidase; EC 1.1.3.10) is a large homotetrameric protein containing one covalently bound FAD per subunit. A crystal structure of POx from T. multicolor was elucidated recently (Hallberg et al., 2004). POx preferrably oxidizes D-glucose, D-galactose, and D-xylose to 2-keto-D-glucose, 2-keto-D-galactose, and 2-keto-D-xylose, respectively, transferring electrons to molecular oxygen but also alternative electron acceptors like 1,4-benzoquinone, 2,6-dichloroindophenol, the ferricenium ion and ferricyanide (Leitner et al., 2001). A catalytically related enzyme, pyranose dehydrogenase (PDH, EC 1.1.99.29), was first isolated from Agaricus bisporus (Volc et al., 1997). It is, like POx, a member of the GMC structural family and is capable of oxidizing a broader variety of monosaccharides, oligosaccharides and glycosides than POx. Contrary to this enzyme, however, PDH is unable to transfer the electrons to dioxygen and is solely dependant on alternative electron acceptors (similar molecules as are also suitable for POx). Its crystal structure was elucidated only recently (unpublished information)
Aims and methods.
The objective of the entire project (two researchers) is to modify the reactivity of the two sugar oxidoreductases POx and PDH with dioxygen as well as alternative electron acceptors. Previous research on the oxygen reactivity of flavin-dependent enzymes has not indicated specific binding sites for either oxygen or alternative electron acceptors, and no clear structural determinants governing whether an enzyme reacts as an oxidase or a dehydrogenase. We therefore propose to use methods of directed evolution as well as semi-rational protein engineering in order to effect changes in reactivity with oxygen, and to identify amino acids and structural properties influencing the oxygen reactivity, in the two enzymes pyranose 2-oxidase and pyranose dehydrogenase. These two enzymes belong to the same structural family, display similarity in the active site and catalyze very similar reactions with respect to the electron donors.
The surface of the cavity that houses the flavin cofactor in in TmPOx and accepts the sugar substrate (si-side) is lined with the amino acids Leu545, Val546, Leu547 and His548, plus Gln448 and Asn593. Val546 interacts with the hydroxyl group at C-1 of glucose. His548 is exposed to the cavity of the active site and is close to the C4a-N5 locus of the flavin. Together with Asn593 it is likely involved in the electron transfer from the sugar substrate to the flavin cofactor (Hallberg et al., 2004, Kujawa et al., 2006).
In AmPDH the corresponding amino acids are Ser534, Tyr535, Val536 and His537, plus Gln433 and His581 (Kujawa et al., 2006). The presence of His537 and His581 provides potentially protonated residues in close proximity to C4a-N5 of the flavin, which would suggest significant oxygen reactivity.
On the re-side side of TmPOx amino acids Thr166, His167, Trp168, Thr169 and Cys170 form a tight loop, which does not leave a lot of space between the flavin moiety and the amino acid surroundings. His167 is the amino acid that is covalently linked to the flavin, and Thr169 is the amino acid most closely in terms of position relative to the flavin cofactor. It is said to be involved in flavin re-oxidation by providing a hydrogen bond to the flavinĀ“s N5-O4, supporting the electron transfer to the acceptor (Kujawa et al. 2006). The corresponding amino acids in AmPDH are Thr127 – His128 – Asn129 – Gly130 – Met131. Gly130 is equivalent to Thr169, which is strictly conserved in all known POx sequences (Kujawa et al. 2006). We plan to systematically investigate these 10 amino acid residues in both enzymes (166 – 170, 448, 545 – 548, 593 in TmPOx; 127 – 131, 433, 534 – 537, 581 in AmPDH) using overlap extension mutagenesis.
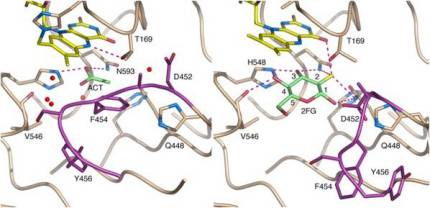
After mutagenesis and propagation the expression vectors will be transformed into the established expression hosts (E. coli BL21 DE3* for TmPOx, P. pastoris X33 for AmPDH). The resulting E. coli and Pichia colonies will be transferred into deep-well microtiter plates containing the appropriate growth medium supplemented with 15% glycerol for cryo-storage. From these master-plates the clones will be inoculated into replica-plates for screening.
Additionally, we propose to use random mutagenesis (by error-prone PCR) to generate libraries of mutant POx and PDH (Arnold and Georgiou, 2003). Randomization will first be limited to the region around the active site, which will increase the scope substantially relative to the semi-rational approach, but will exclude regions where an effect on the reactivity in general is not to be expected. We will therefore first target a region in both enzymes comprising just less than a third of the enzyme, from position 440 (TmPOx) and 420 (AmPDH), respectively, to the C-terminus.
Screening of POx variants will be done using the standard oxygen (ABTS)-based assay. In parallel an assay will be run using ferricenium as alternative electron acceptor (Kujawa et al., 2007). The comparison of these two assays will enable the identification of variants whose oxygen reactivity has decreased substantially or been abolished, but whose activity with ferricenium remains unaltered. Screening of PDH variants will be done analogously, allowing for longer reaction times in the oxygen (ABTS)-based assay in order to detect any activity at all. A parallel standard assay with ferricenium as electron acceptor will be done to identify enzyme variants with reduced reactivity with this acceptor, even if no catalytic activity with oxygen can be detected. New identified POx and PDH variants will be checked by sequencing, purification and characterization. Transient kinetics of the reductive half-reaction (carbohydrate oxidation) and the oxidative half-reaction (reduction of electron acceptors) will be measured under both aerobic and anaerobic conditions using a stopped-flow spectrophotometer. Mutations with a desired effect (reduced or eliminated oxygen reactivity plus unaltered reactivity with alternative electron acceptors for POx; measurable catalytic activity with oxygen in the absence of any alternative acceptor for PDH) in either of the approaches will be combined by site-directed mutagenesis, followed by characterization of the respective double-, triple- and multiple-mutants as described above. Special focus will be on stopped-flow spectrophotometry in order to distinguish between influences caused by the interaction of the enzyme with the sugar substrate, and influences that affect the re-oxidation of the reduced flavin by the electron acceptor.
Arnold FH, Georgiou G (Eds.) (2003) Directed Evolution Library Creation Methods and Protocols, Totowa, New Jersey, Humana Press.
Hallberg BM, Leitner C, Haltrich D, Divne C (2004) Crystal structure of the 270 kDa homotetrameric lignin-degrading enzyme pyranose 2-oxidase. J Mol Biol, 341, 781-96.
Joosten V, Van Berkel WJ (2007) Flavoenzymes. Curr Opin Chem Biol, 11, 195-202.
Kass IJ, Sampson NS (1998) Evaluation of the role of His447 in the reaction catalyzed by cholesterol oxidase. Biochemistry, 37, 17990-18000.
Kujawa M, Volc J, Halada P, Sedmera P, Divne C, Sygmund C, Leitner C, Peterbauer C, Haltrich D (2007) Properties of pyranose dehydrogenase purified from the litterdegrading fungus Agaricus xanthoderma. FEBS J, 274, 879-894.
Kujawa M, Ebner H, Leitner C, Hallberg BM, Prongjit M, Sucharitakul J, Ludwig R, Rudsander U, Peterbauer C, Chaiyen P, Haltrich D, Divne C (2006) Structural basis for substrate binding and regioselective oxidation of monosaccharides at C3 by pyranose 2-oxidase. J Biol Chem, 281, 35104-35115.
Leferink NG, Fraaije MW, Joosten HJ, Schaap PJ, Mattevi A, Van Berkel WJ (2009) Identification of a gatekeeper residue that prevents dehydrogenases from acting as oxidases. J Biol Chem, 284, 4392-4397.
Leitner C, Volc J, Haltrich D (2001) Purification and characterization of pyranose oxidase from the white rot fungus Trametes multicolor. Appl Environ Microbiol, 67, 3636-44.
Mattevi A (2006) To be or not to be an oxidase: challenging the oxygen reactivity of flavoenzymes. Trends Biochem Sci, 31, 276-283.
Volc J, Kubatova E, Wood DA, Daniel G (1997) Pyranose 2-dehydrogenase, a novel sugar oxidoreductase from the basidiomycete fungus Agaricus bisporus. Arch Microbiol, 167, 119-25.
Volc J, Kubatova E, Wood DA, Daniel G (1997) Pyranose 2-dehydrogenase, a novel sugar oxidoreductase from the basidiomycete fungus Agaricus bisporus. Arch Microbiol, 167, 119-25.